Detection of biomagnetic fields from Venus flytrap plants
When living plants are stimulated, they produce electrical signals that can travel within a cellular network analogous to the animal nervous system. By monitoring voltage changes in plant cells and organs, biologists have identified two types of electrical signaling in plants: all-or-nothing action potentials (APs) similar to those seen in animal cells, and weaker slow-wave potentials (SWPs) which can last up to several minutes. While SWPs are found in most plant species, APs are restricted to unique species like the “sensitive plant”, Mimosa pudica, and the carnivorous Venus flytrap, Dionaea muscipula. In collaboration with the University of Würzburg Biocenter and the Physikalisch-Technische Bundesanstalt (PTB) Berlin, we asked the question: is electrical activity in the Venus flytrap accompanied by magnetic signals?
Although human and animal biomagnetism are well-developed areas of study [1-6], very little equivalent work has been conducted in the plant kingdom. Previously reported detection of plant biomagnetism was carried out using superconducting-quantum-interference-device (SQUID) magnetometers [7-9]. Our research uses atomic magnetometers, which employ a glass cell containing alkali vapor to sense changes in the local magnetic-field environment [10-12]. Atomic magnetometers are arguably more attractive than SQUIDs for biological applications, since they are non-cryogenic and can be miniaturized to optimize spatial resolution.
The bilobed trap of the Dionaea plant (Fig. 1a) contains mechanosensitive hairs which, when touched, activate the firing of an AP that travels throughout the trap [13,14]. After two consecutive APs, the trap closes, imprisoning a possible prey insect for subsequent digestion. The trap is electrically excitable by a variety of energy inputs—not only mechanical (touch or wounding), but also osmotic (salt water) and thermal (heat or cold). Under prolonged heat stimulation over 34°C, traps can enter an autonomous AP firing mode, as intracellular calcium levels oscillate around the threshold required for AP release.
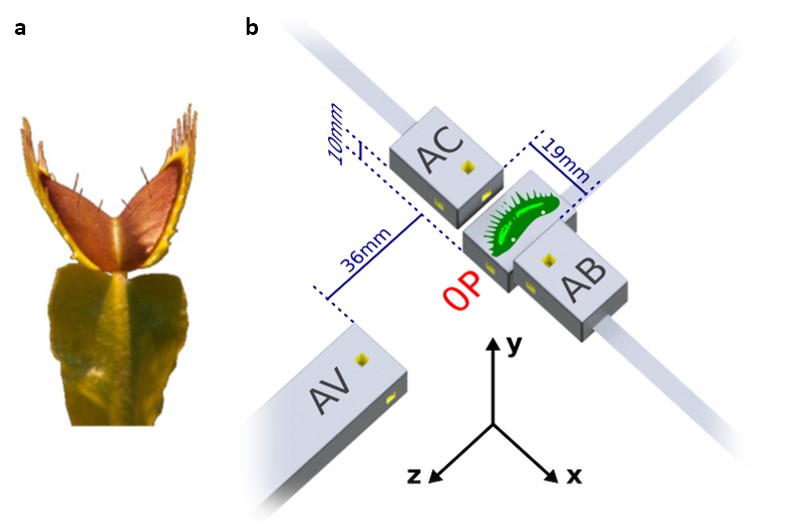
Fig. 1. Venus flytrap (a) and magnetometry setup (b).
Flytrap experiments were carried out at PTB Berlin in the Berlin Magnetically Shielded Room 2 (BMSR-2) facility [15] with the setup shown in Fig. 1b. An isolated trap lobe was attached to the housing of the primary magnetometer (denoted 0P), such that the sample offset from the center of the 3×3×3-mm3 rubidium sensing volume (yellow) was approximately 7 mm. Three additional magnetometers were placed nearby to monitor signal fall-off as well as the magnetic background in the shielded room. To simultaneously record APs, we inserted two surface electrodes in either end of the plant sample (locations indicated by white dots); careful tests were performed to ensure that no spurious magnetic fields were generated by the electrode system. Resistive heaters in the magnetometer housing were used to increase the atomic density and improve sensitivity, as well as to induce autonomous AP firing via surface heat transfer. Magnetic signals were observed only in the data of magnetometer 0P. To minimize common background noise, we subtracted the magnetic data of magnetometer AV to create a gradiometer with 48-mm baseline.
Results from several different experiments are shown in Fig. 2. The plots were obtained by triggering on the measured electric signals and averaging the magnetic data in a time window around those trigger points. Signals of up to 0.5 pT are visible in the y-axis
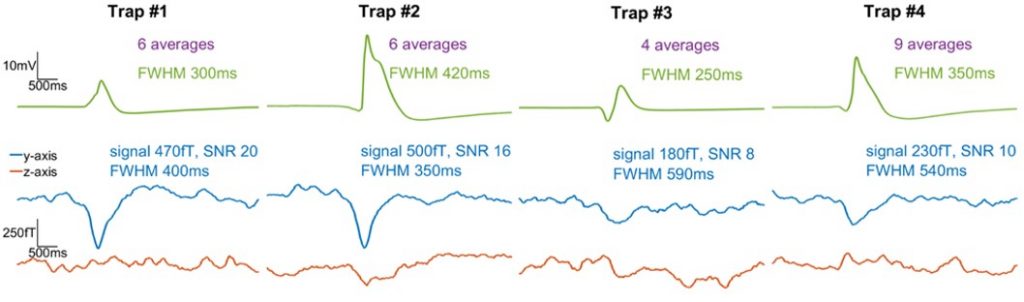
Fig. 2. Comparison of average electric and gradiometric signals from four different plant samples.
One outstanding question in plant electrophysiology is how electrical signals propagate over long distances through the plant system. A proposed pathway of long-distance communication between plant cells is electrically conductive phloem in the vasculature. Phloem is a living tissue which also serves to transport photosynthates. Based on the magnitude and directionality of the measured magnetic signals, as well as the known vasculature structure of the trap, our experimental results support the hypothesis that vasculature acts as a network for long-distance electrical signaling in the Venus flytrap.
In recent decades, noninvasive magnetic techniques have become essential tools for medical diagnostics of the human brain and body. By optimizing the low-frequency stability and sensitivity of plant magnetometers, we hope to apply this technology in the future for crop-plant diagnostics—for example, measuring the electromagnetic response to such external challenges as fertilizer deprivation, disease, and global warming. At the same time, the Venus flytrap is a promising system for renewable-energy research, since the traps naturally accept and store various forms of energy input for direct conversion into electrical energy.
Text adapted from:
A. Fabricant, G. Iwata, S. Scherzer, L. Bougas, K. Rolfs, A. Jodko-Władzińska, J. Voigt, R. Hedrich, and D. Budker, “Action potentials induce biomagnetic fields in carnivorous Venus flytrap plants” [manuscript in preparation].
References:
- S. Baillet, “Magnetoencephalography for brain electrophysiology and imaging”, Nat. Neurosci. 20, 327-339 (2017).
- D. Cohen, “DC magnetic fields from the human body generally: a historical overview”, Neurol. Clin. Neurophysiol. 2004, 93 (2004).
- S. J. Williamson, G. L. Romani, L. Kaufman, and I. Modena (Eds.), Biomagnetism: An Interdisciplinary Approach (Springer US, 1983).
- K. Jensen, R. Budvytyte, R. A. Thomas, T. Wang, A. M. Fuchs, M. V. Balabas, G. Vasilakis, L. D. Mosgaard, H. C. Stærkind, J. H. Müller, T. Heimburg, S. P. Olesen, and E. S. Polzik, “Non-invasive detection of animal nerve impulses with an atomic magnetometer operating near quantum limited sensitivity”, Sci. Rep. 6, 29638 (2016).
- K. Jensen, M. A. Skarsfeldt, H. Stærkind, J. Arnbak, M. V. Balabas, S. P. Olesen, B. H. Bentzen, and E. S. Polzik, “Magnetocardiography on an isolated animal heart with a room-temperature optically pumped magnetometer”, Sci. Rep. 8, 16218 (2018).
- J. F. Barry, M. J. Turner, J. M Schloss, D. R. Glenn, Y. Song, M. D. Lukin, H. Park, and R. L. Walsworth, “Optical magnetic detection of single-neuron action potentials using quantum defects in diamond”, PNAS 201601513 (2016).
- Z. Trontelj, R. Zorec, V. Jazbinšek, and S. N. Erné, “Magnetic detection of a single action potential in Chara corallina internodal cells”, Biophys. J. 66, 1694-1696 (1994).
- V. Jazbinšek, G. Thiel, W. Müller, G. Wübbeler, and Z. Trontelj, “Magnetic detection of injury-induced ionic currents in bean plants”, Eur. Biophys. J. 29, 515-522 (2000).
- F. Baudenbacher, L. E. Fong, G. Thiel, M. Wacke, V. Jazbinšek, J. R. Holzer, A. Stampfl, and Z. Trontelj, “Intracellular axial current in Chara corallina reflects the altered kinetics of ions in cytoplasm under the influence of light”, Biophys. J. 88, 690-697 (2005).
- D. Budker and M. Romalis, “Optical magnetometry”, Nat. Phys. 3, 227-234 (2007).
- E. Corsini, V. Acosta, N. Baddour, J. Higbie, B. Lester, P. Licht, B. Patton, M. Prouty, and D. Budker, “Search for plant biomagnetism with a sensitive atomic magnetometer”, J. Appl. Phys. 109, 074701 (2011).
- J. Osborne, J. Orton, O. Alem, and V. Shah, “Fully integrated, standalone zero field optically pumped magnetometer for biomagnetism”, Proc. SPIE 10548, Steep Dispersion Engineering and Opto-Atomic Precision Metrology XI, 105481G (2018).
- J. Böhm, S. Scherzer, E. Krol, I. Kreuzer, K. von Meyer, C. Lorey, T. D. Mueller, L. Shabala, I. Monte, R. Solano, K. A. S. Al-Rasheid, H. Rennenberg, S. Shabala, E. Neher, and R. Hedrich, “The Venus flytrap Dionaea muscipula counts prey-induced action potentials to induce sodium uptake”, Curr. Biol. 26, 286-295 (2016).
- A. G. Volkov, “Signaling in electrical networks of the Venus flytrap (Dionaea muscipula Ellis)”, Bioelectrochemistry 125, 25-32 (2019).
- F. Thiel, A. Schnabel, S. Knappe-Grüneberg, D. Stollfuß, and M. Burghoff, “Demagnetization of magnetically shielded rooms”, Rev. Sci. Instrum. 78, 035106 (2007).